We make thousands of decisions every day. It has been estimated that we make around 220 decisions each day only related to food (1). Choice is fundamental for our lives, as well as for the behaviour of all the other animals. Making a decision requires to integrate physiological and psychological information to choose a course of action or a belief among several available options.
Understanding the mechanisms by which animals integrate information from their senses over space and time to make sensory-motor decisions is at the core of the reviewed article of this month: Neural circuits for evidence accumulation and decision making in larval zebrafish, published in Nature Neuroscience in January 2020 (2).
In this article, we will briefly introduce the concept of integrator models (which have been proposed to explain how the brain process the information to make a choice), we will illustrate the experimental approaches of the reviewed paper, and we will state the results they obtained.
Integrating the information of the environment: how can we model it?
Sensory information is generally obscured by noise, it is often ambiguous and conflicting. Animals need to constantly integrate information from their senses over space and time to resolve such ambiguities in order to infer the actual state of the world and make a decision. A fruitful approach to study how the brain processes this entangled information relates to mathematical models of evidence integration (3).
These mathematical models provide a framework for using behavioural measurements to infer a subject’s decision strategy. One example of these models are drift-diffusion models, for simple, two-choice decision processes. These models postulate that decisions are made by a noisy process that accumulates information over time until a threshold is surpassed or until the stimulus disappears, at which point a decision is made toward one of two responses criteria (4).
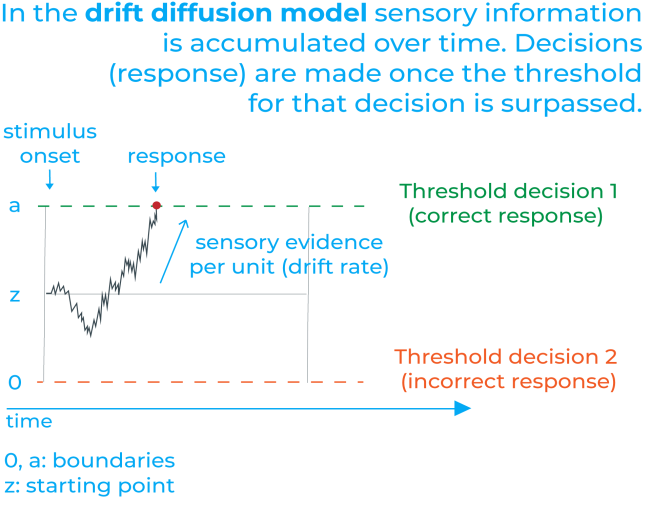
In the drift diffusion model, the distance between the starting point and each threshold represents the amount of information needed to commit to the different response alternatives. As evidence favouring one alternative is collected, the signal accumulates toward the corresponding threshold (4).
When the decision process reaches a threshold, the decision is made. The critical parameter that determines whether the decision emphasizes speed or accuracy is the height of the decision threshold. A high threshold results in few errors, but slow reactions, whereas a low threshold results in more errors, but faster reactions. The difficulty of the task and the perceptual abilities of the subject are reflected in the drift parameter. An easy task will have a higher drift rate than a hard one, while subjects who are skilled at the task will have higher drift rates than subjects who are not (4).
Random dot motion kinematograms
A classical kind of stimuli where the drift-diffusion model has been applied are random dot kinematograms, where two or more patterns of random dots are presented in sequence (5). These patterns of dots are composed by a fraction of flickering dots that moves coherently over the screen and the remaining elements which are randomly redrawn across the visual field (2).
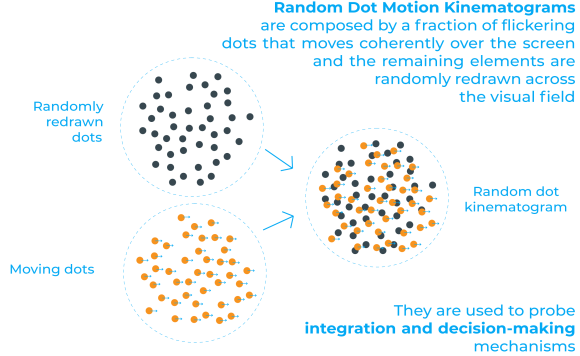
This kind of stimuli have already been used to probe integration and decision-making mechanisms in the visual field of primates, and in the reviewed paper these stimuli have been applied to zebrafish.
Several are the benefits of random dots to dissect the neuronal basis of spatiotemporal integration. For example, dots have short lifetimes, which allows animals to track individual objects over the time course of stimulation. Besides, in this type of experiments, it is possible to set different levels of coherence between the flickering random dots. This allows to regulate the stimulus strength without changing luminance, contrast, or speed (2).
Studying sensory-motor decisions in zebrafish larvae
To study whether larval zebrafish can accumulate sensory evidence and use those signals for decision making, in the reviewed paper they projected random dot motion stimuli onto the floor of an arena containing freely swimming larvae (2).
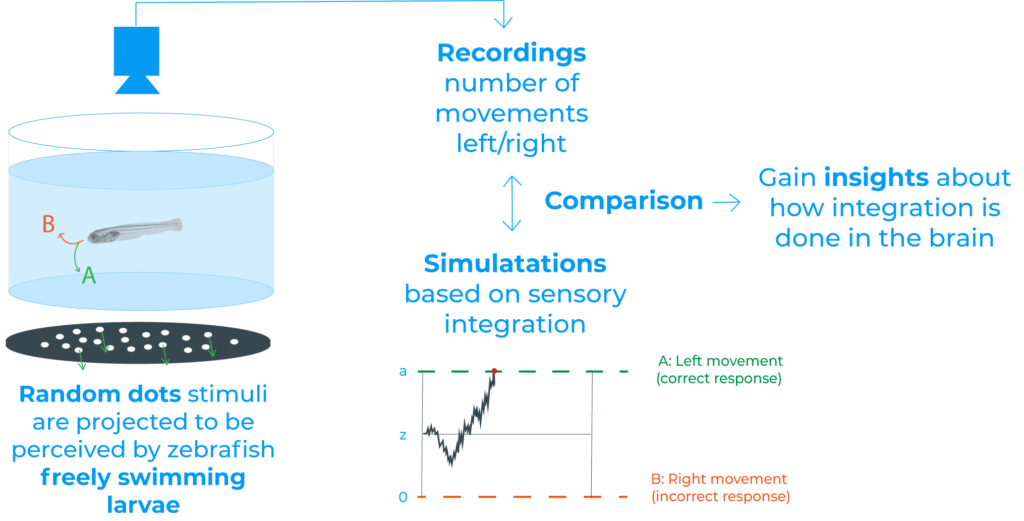
For an easier understanding we recommend to watch the following video, which is the Supplementary Video 1 of (2). In the video we see freely swimming larval zebrafish with example dot motion stimulus:
After tracking animal behaviour, they found that larvae robustly followed coherent motion direction (2). Moreover, they concluded that decisions seem to be based on some form of sensory integrations, since the accuracy of larval movements improved over time and over consecutive swims, among other results (2).
Additionally, they also wanted to explore how and where in the brain this integration takes place. To that end, they used brain-wide two-photon calcium imaging (2). This technique allowed them to screen for areas whose temporal dynamics are aligned with the integration proposed.
Two-photon calcium imaging
Calcium ions are responsible and generate a wide variety of intracellular signals. In the nervous system, calcium ions are present at both pre- and postsynaptic levels and related to a large collection of functions such as the release of neurotransmitter, neuronal plasticity, and gene expression (6).
Two-photon imaging was developed in the early 1990s (7) and was used for the first time for calcium imaging in the nervous system in the mid-1990s (8,9). Since then, two-photon imaging has revolutionized calcium imaging and is now used worldwide as a powerful technique to monitor the activity of distinct neurons in brain tissue in vivo.
In two-photon imaging, two low-energy photons (typically from the same laser) cooperate to produce a transition from the ground to the excited state in a fluorescent molecule (fluorophore). This two-photon effect must occur within a femtosecond time window. Due to this cooperation, the process of absorption is non-linear and, as a result excitation occurs in a tiny focal volume. The key consequence of localization of excitation is three-dimensional contrast and resolution (10,11).
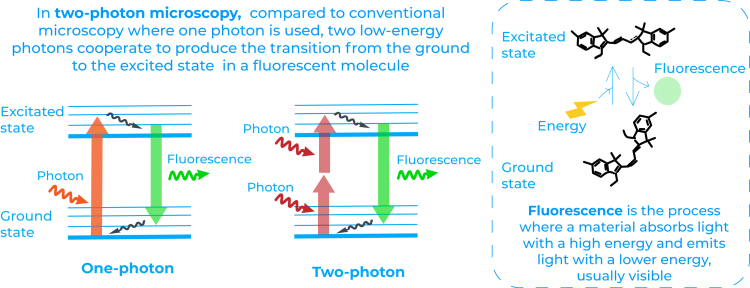
Besides, the excitation wavelengths used in two-photon imaging are mostly deep red and near infrared, which penetrate the tissue better than the visible wavelengths used in one-photon microscopy. Thus, making it possible to image thicker tissues (10,11).
Defining brain circuits in zebrafish
In the reviewed work, they embedded zebrafish larvae in transparent agarose and imaged the larval brains with two-photon calcium imaging while larvae were exposed to random dot stimuli (2). Larvae were either full- or half-embedded (head-fixed and tail free). The latter allowing to also analyse larval behaviour at the same time.
Their results suggest that the pretectum performs the spatial pooling of local motion cues whereas the anterior hindbrain temporally integrates these signals. As the authors state, both ideas agree with recent studies in zebrafish that defend that the pretectum computes direction-selective global motion signals and that the anterior hindbrain is a centre of sensory-motor convergence (2).
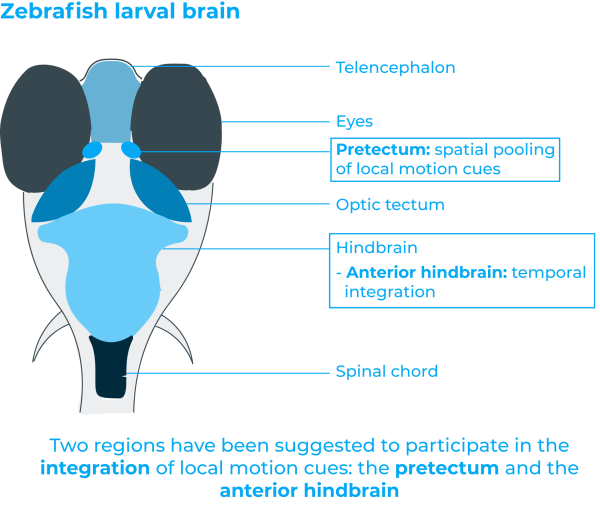
Finally, and more interestingly, they propose a biophysically plausible circuit model in the anterior hindbrain that can describe how freely behaving animals accumulate evidence from a complex sensory environment and interact with it.
Conclusion
Decision-making is a complex process, where many aspects are considered. In sensory-motor decisions, animals need to constantly integrate the information perceived by their senses over space and time. In the reviewed paper, zebrafish larvae have been used as a model to study the brain circuitry and mechanisms underlying the decision-making process responsible for the optomotor response. This behaviour appears when larvae are stimulated with global motion drift, since larvae follow the overall motion direction after that stimulus.
They provide the first evidence that larval zebrafish can temporally integrate noisy coherent motion stimuli over many seconds and suggest a model for the integration and the brain parts implicated.
It is interesting to point out that thanks to its methodology the reviewed work provides powerful toolkits to study sensory integration and decision-making in zebrafish larvae. This creates new opportunities to analyse precisely the neural process underlying such behaviours, which could also help to uncover other principles underlying sensory evidence accumulation and decision making in other organisms.
Shared if you liked it!
References
- Wansink B, Sobal J. (2017) mindless eating: the 200 daily food decisions we overlook. Environment and Behavior, https://doi.org/10.1177/0013916506295573
- Bahl, A., Engert, F. (2020). Neural circuits for evidence accumulation and decision making in larval zebrafish. Nature neuroscience, https://doi.org/10.1038/s41593-019-0534-9
- Stine, G. M., Zylberberg, A., Ditterich, J., Shadlen, M. N. (2020). Differentiating between integration and non-integration strategies in perceptual decision making. eLife, https://doi.org/10.7554/eLife.55365
- Ratcliff, R., McKoon, G. (2008). The diffusion decision model: theory and data for two-choice decision tasks. Neural computation, https://doi.org/10.1162/neco.2008.12-06-420
- Random dot kinematogram. Oxford Reference. Retrieved in August 2021. https://www.oxfordreference.com/view/10.1093/oi/authority.20110803100403766
- Grienberger, C., Konnerth, A. (2012). Imaging calcium in neurons. Neuron, https://doi.org/10.1016/j.neuron.2012.02.011
- Denk, W., Strickler, J. H., & Webb, W. W. (1990). Two-photon laser scanning fluorescence microscopy. Science, https://doi.org/10.1126/science.2321027
- Denk, W., Delaney, K. R., Gelperin, A., Kleinfeld, D., Strowbridge, B. W., Tank, D. W., & Yuste, R. (1994). Anatomical and functional imaging of neurons using 2-photon laser scanning microscopy. Journal of neuroscience methods, 54(2), 151–162. https://doi.org/10.1016/0165-0270(94)90189-9
- Svoboda, K., Denk, W., Kleinfeld, D., & Tank, D. W. (1997). In vivo dendritic calcium dynamics in neocortical pyramidal neurons. Nature, 385(6612), 161–165. https://doi.org/10.1038/385161a0
- Ellis-Davies G. C. (2011). Two-photon microscopy for chemical neuroscience. ACS chemical neuroscience, https://doi.org/10.1021/cn100111a
- Svoboda, K., Yasuda, R. (2006). Principles of two-photon excitation primer microscopy and its applications to neuroscience. Neuron, https://doi.org/10.1016/j.neuron.2006.05.019